Introduction
Severe Acute Respiratory Syndrome- Coronavirus disease 2 (SARS-CoV-2) belonging to the family Coronaviridae caused a massive pandemic Coronavirus Diseases (COVID-19) between 2019 and 2022 worldwide. It is a beta coronavirus derived from bats that causes severe respiratory disease or disorder (Ciotti et al., 2020). In December 2019, the first case of COVID-19 was reported in Wuhan, China, and the virus was given the name severe acute respiratory syndrome coronavirus 2 or SARS-CoV 2. In March 2020, the World Health Organization (WHO) declared coronavirus to be a pandemic (Yuki, Fujiogi, & Koutsogiannaki, 2020) More than 767 million cases and over 6 million fatalities have been reported as of July 12, 2023 (WHO, 2023). Maximum genetic identity exists between SARS-CoV 2 and the 2003-spreading "severe acute respiratory syndrome coronavirus 1" as well as the 2012-spreading Middle East Respiratory Syndrome (MERS). It is also 96.3% identical to the horseshoe bat coronavirus and 82% identical to the human SARS coronavirus 1. The virus undergoes rapid mutations resulting in the emergence of numerous variants which are further classified into variants of concern (VOC), variants of interest (VOI), variants being monitored (VBM), and variants of high consequences (VOHC) (Chavda, Mishra, & Vuppu, 2022).
Various strategies were followed by the governments of different countries to tackle this pandemic. An important approach is the endurance of proper COVID behavior. As SARS-CoV-2 is an airborne pathogen, wearing face masks and following social distancing in crowded places is of utmost importance. Hand hygiene must be obeyed whenever somebody steps out or even touches any suspicious substances. Hand washing with water and soap and the use of hand sanitizers are two crucial methods of hand hygiene. Numerous health professionals from around the world have authorized and helped the use of hand sanitizers. Hand sanitizers eliminate SARS-CoV-2 from hand surfaces and break the transmission chain efficiently (Prajapati, Desai, & Chandarana, 2022). Excessive care must be taken upon touching doorknobs, buttons in elevators, newspapers, fruits, and vegetables that enter the house from outside exposure (Greenhalgh et al., 2021; Joshi & Mehendale, 2021). Another important preventive strategy is isolation and quarantining. With the disease spreading rapidly, several measures were taken including self-isolation. People were quarantined at their houses. However, this strategy proved to be quite limited due to the large occurrence of asymptomatic individuals (Joshi & Mehendale, 2021).
Vaccination is an important preventive strategy. Vaccines have been developed as a biological measure to prevent COVID-19 alongside behavioral measures. Though the relative roles of humoral and cellular responses in vaccine effectiveness are still being studied, the rapid development of vaccines using new technologies is an impressive scientific achievement. Promising results from early and interim trials have shown various vaccines' safety, immunogenicity, and efficacy (Lazarus et al., 2023). Vaccination is necessary in building herd immunity and curtailing the spread of the virus, as demonstrated in countries like the USA, Israel, and the UK. Vaccination, even though not 100% effective, reduces the severity of COVID-19 cases. However, it is important to address vaccine hesitancy and concerns through research-based evidence (Dagan et al., 2021; Greenhalgh et al., 2021).
Additional development of novel therapeutic approaches with greater efficacy than conventional therapeutics is also a crucial approach in COVID-19 management. One such approach is the utility of plant-based derivatives for treatment targeted to the affected organ.
Phytochemicals are bioactive compounds found in plants that have potential therapeutic properties. When combined with nanoparticles, they provide numerous benefits, particularly in the area of targeted drug delivery and enhanced therapeutic efficacy (Hussain et al., 2022). The use of nanoparticles as carriers for phytochemicals allows for improved solubility, stability, and controlled release of these compounds, enhancing their bioavailability and therapeutic effects.
Nanotechnology has been shown to greatly influence cancer treatment. Phytochemicals targeted with nanoparticles can help in selectively target the cancerous cells in the body, delivering the therapeutic agents to the locus of disease, and consequently also help in improving the effectiveness of the chemotherapeutic drugs. The formation of nanoparticles can alter the way phytochemicals are distributed and processed in the body, leading to improved therapeutic outcomes. This change in pharmacokinetics and biodistribution helps enhance the therapeutic index of phytochemicals by reducing their toxicity and increasing their effectiveness (Xie et al., 2016). Certain phytochemicals loaded as nanoparticles can retain anti-inflammatory properties that inhibit the pro-inflammatory properties and immunomodulation responses. This may be beneficial to treat chronic inflammatory disorders like rheumatoid arthritis, inflammatory bowel disease, and asthma (Dhamodharan & Mirunalini, 2013). Phytochemicals with antioxidant properties, when loaded onto nanoparticles, can also offer enhanced protection against oxidative stress. They can scavenge free radicals, reduce oxidative damage, and potentially slow down the aging process. This application can be beneficial in age-related diseases and conditions associated with oxidative stress (Vaiserman, Koliada, Zayachkivska, & Lushchak, 2020). A few phytochemicals loaded with nanoparticles are known to demonstrate neuroprotective effects. They have the ability to cross the blood-brain barrier, shield neurons from injury, and may be used to prevent or cure neurodegenerative illnesses including Alzheimer’s and Parkinson’s (Paramanick, Singh, & Singh, 2022). Phytochemical-loaded nanoparticles can promote wound healing by accelerating tissue regeneration, reducing inflammation, and preventing infection. They can be used in the development of advanced wound dressings and topical formulations (Qadir et al., 2021). Additionally, some phytochemicals loaded with nanoparticles have shown the potential to improve cardiovascular health. They can help in reducing cholesterol levels, preventing plaque formation, and improving vascular function, thus reducing the risk of cardiovascular diseases (Banerjee, 2020). Furthermore, several phytochemicals are known to exhibit antibacterial activity against a variety of harmful microorganisms such as viruses, bacteria, and fungi.
Phytochemicals have long been thought to be promising candidates for identifying therapeutic molecules to treat complex disorders, such as viral infections and their consequences. Among these problems is lung damage, which is induced by the inflammatory response elicited by SARS-CoV-2. Various phytochemicals that exhibit protective effects against lung injuries induced by various methods, as well as their underlying pharmacological mechanisms, such as cepharanthine, epigoitrin, isofraxidin, osthole, resveratrol, apigenin, kaempferol, myricetin, quercetin, chlorogenic acid, chicoric acid, emodin, thymoquinone, (Sandoval-Gallegos et al., 2021). However, despite the efficacy of these natural compounds in combating viral diseases, it is important to overcome their pharmacokinetic limitations through the development of novel drug delive potential discovery of alternative drugs with anti-viral effects that can effectively control the complications associated with COVID-19. furtherrrequired to establish the exact dysregulated pathways in COVID-19 and the possible impacts of phytochemicals on human beings.
Phytochemical-loaded Nanoparticles
Plants are source of phytochemicals that exhibit various pharmacological properties like anti-inflammatory, anti-tumor, anti-oxidant, antimicrobial, or anti-genotoxicity (Koklesova et al., 2023). The phytochemicals are also reported to be used as immunostimulant for treatment of many diseases. These secondary metabolites are advantageous in regulation of natural body processes. The therapeutic activity of the phytocompounds involves interaction with several pathways causing inhibition of overexpression of proteins, or influencing the hormone and enzyme activity. The therapeutic efficacy of phytocompounds is mediated by interactions with numerous pathways, which result in the suppression of protein overexpression or the influence of hormone and enzyme function (More et al., 2021). The therapeutic phytochemicals can be utilized in the domain of nanomedicine to develop drugs and vaccines against numerous medical conditions like cancers, viral and bacterial infections, etc. The efficacy however, can be reduced attributed to their low bioavailability on oral consumption and low solubility in water (Koklesova et al., 2023). The encapsulation of the phytochemicals in nanostructures enhances its efficacy by promoting targeted delivery to the specific location in the human body (Chavda et al., 2023).
Historical Account
Medicinal plant resources serve as a source of medicine since ancient times. These are obtained from various parts like leaves, stems, fruits, and roots (Armendáriz-Barragán et al., 2016). Their metabolites, especially the secondary metabolites such as alkaloids, phenolic acid, saponins, indoles, isothiocyanates, phytosterols, and furans (Khan, Karnpanit, Nasar-Abbas, Huma, & Jayasena, 2015; Pinciroli et al., 2018), not only have an advantage for the plant’s protection but also aid in health benefits to humankind. The complicated amalgam of substances derived provides numerous benefits like antiviral, anticancer, antifungal, antioxidant, etc. (Husni & Ramadhania, 2021). Medicinal herbs have been used for centuries to treat diseases. This is supported by the evidence from cave carvings and literature, as well as the number of medicinal plants that are listed in Ayurvedic and traditional Chinese medicine texts (Sarker & Nahar, 2013; Shakya, 2016). The first studies on phytochemicals-loaded nanoparticles were conducted in the early 1990s. In 1991, a study showed that nanoparticles could be used to deliver curcumin, a phytochemical with anti-inflammatory properties, to cells in the brain (Aggarwal, Kumar, & Bharti, 2003). In the following years, several studies investigated the use of nanoparticles to deliver other phytochemicals, such as quercetin, resveratrol, and genistein. These studies showed that nanoparticles could improve the bioavailability of phytochemicals and increase their therapeutic potential (Chen, Roy, Yang, & Prasad, 2016; Khan, Saeed, & Khan, 2019).
Some crude extract of phytochemicals obtained from medicinal plants contain cytotoxic compounds (Thomas, Joy, Mathew, & Skaria, 1999). Proper extraction, purification, and characterization of the phytochemicals are to be made for better usage of conventional drugs. These drugs are highly biocompatible, bioactive, and bioavailable for effective drug activity (Ajazuddin & Saraf, 2010). As these phytochemicals pass through the absorption, digestion, and biochemical conversion process, the bio-accessibility and bioavailability of these phytochemicals are minimal (Karthik et al., 2015; Oehlke et al., 2014; Rein et al., 2013). These may be not able to be absorbed into the bloodstream effectively, which can limit their therapeutic potential (Conte et al., 2016). The stability of these phytochemicals also plays a vital role in their efficacy as these can be sensitive to heat, light, and moisture. Degradation of these can lead to loss of potency and formation of byproducts (Conte et al., 2016). Thus, nanoencapsulation technology, such as nanoparticles, can provide an alternative for the effective delivery of these bioactive compounds without compromising their bio-efficacy and reduce the toxic effects of these compounds at optimal concentrations (Ahmad, Srivastava, Ghosh, & Khare, 2021).
Recently, many studies regarding the use of nanotechnology-based systems have been formulated like nanoparticles (NPs), liposomes, and nanoemulsions (Zorzi, Carvalho, Poser, & Teixeira, 2015). Among these, the small-sized nanostructure of these nanoparticles provides a suitable medium for the phytochemicals to be delivered into the body due to their free movement (Zeeshan, Farhan, & Siddiqui, 2014). The use of inorganic nanoparticles such as mesoporous silica nanoparticles, superparamagnetic Iron-Oxide Nanoparticles (SPIONs), and gold/silver nanoparticles provides poor drug loading efficacy and high peripheral toxicity (Lombardo, Kiselev, & Caccamo, 2019). In contrast, organic nanoparticles, such as lipid-based drug delivery systems, protect the drug from degradation and prevent organ toxicity. Consequently, this contributes to the therapeutic index and site-specific target of the drug (Khan et al., 2015). The increasing stability of the phytochemicals is provided by NPs from biological (like enzymes, and pH), environmental (like light, and temperature), and degradation or inactivation (Kamel, Khalil, Rateb, Elgendy, & Elhawary, 2017). Lipid-based delivery systems, such as nanostructure liposomes and nanoemulsions, can be used to increase the solubility of phytochemicals. This is because these systems can encapsulate phytochemicals in a protective layer, which helps to prevent them from degrading and also allows them to be targeted to specific cells or tissues (Gupta, Kesarla, & Omri, 2013; Mouhid et al., 2017). Figure 1 presents some common classes of phytochemicals and nanoparticles involved in the phytochemical-loaded nanoparticle systems. When loaded into liposomes, anticancer compounds like vinorelbine and curcumin have a prolonged half-life and fewer side effects, according to research. This is because the liposomes protect the compounds from degradation and also help to target them to cancer cells (Chen, Liu, Zhao, Wang, & Feng, 2012; Wang et al., 2018). The main nanoparticles formulation used for plant extract are nanoliposomes, solid lipid nanoparticles (SLN), silica NPs, polymeric NPs, etc. Among these, polymeric-based nanoparticles and poly (lactic-co-glycolic) acid (PLGA) are widely used (Husni & Ramadhania, 2021).
Development of phytochemical-loaded nanoparticles
The development and preparation of phytochemical-loaded nanoparticles is a complex process that involves the selection of the phytochemical and the nanoparticle system, as well as the preparation, the characterization, and the in vitro/in vivo evaluation of the nanoparticles (Armendáriz-Barragán et al., 2016).
The selection of the phytochemical, nanoparticle system and preparation of the nanoparticles are all important steps in the development of phytochemicals loaded nanoparticles. The phytochemical should have the desired therapeutic properties and be stable in the nanoformulation. This means that the phytochemical should not degrade when it is encapsulated in the nanoparticles. Some factors that can affect the stability of phytochemicals in nanoparticles include the pH of the solution, the presence of other molecules, and the temperature (Zorzi et al., 2015). The nanoparticle system should be compatible with the phytochemical and able to protect it from degradation. The nanoparticle system should also be able to deliver the phytochemical to the target cells or tissues in the body. Some factors that can affect the compatibility of a nanoparticle system with a phytochemical include the size and shape of the nanoparticles, the surface charge of the nanoparticles, and the hydrophobicity of the phytochemical. The nanoparticles are then prepared using a variety of methods, such as solvent evaporation or emulsion polymerization. The characterization of the nanoparticles is also important to ensure that they have the desired properties, such as size, shape, and surface charge (Shakeri & Sahebkar, 2016). This is done using a variety of techniques, such as dynamic light scattering, transmission electron microscopy, and Zeta potential measurement. The size and shape of the nanoparticles can affect their stability and their ability to deliver the phytochemical to the target cells or tissues (Kamel et al., 2017; Shakeri & Sahebkar, 2016). The surface charge of the nanoparticles can also affect their stability and their ability to interact with the target cells or tissues. Several factors can affect the encapsulation efficiency of an extract, including the type of extract, the type of nanoparticle system, and the method used to prepare the nanoparticles. Typically, the encapsulation efficiency of an extract is determined using a method called UV-vis spectroscopy. This method measures the amount of extract that is present in the nanoparticles and in the supernatant (the liquid that is not encapsulated in the nanoparticles). The release profile of an extract from the nanoparticles is an important parameter to consider when developing phytochemical-loaded nanoparticles (Zorzi et al., 2015). A controlled release profile is desirable because it ensures that the extract is released at a rate that is consistent with the needs of the treatment. The release study of polyphenols from NPs was shown to be 20% at pH 1.2 and 80% at pH 7.4, respectively (Sanna et al., 2015).
Finally, the nanoparticles are evaluated in vitro and in vivo to assess their efficacy and safety. In vitro studies can be used to assess the ability of the nanoparticles to deliver the phytochemical to the target cells or tissues and to assess the toxicity of the nanoparticles. In its turn, in vivo studies can be used to assess the efficacy of the nanoparticles in a living organism and to assess the long-term toxicity of the nanoparticles (Kamel et al., 2017; Shakeri & Sahebkar, 2016; Zorzi et al., 2015).
Mechanism of Action
Phytochemicals are naturally occurring compounds found in plants that have a variety of biological activities, including antioxidant, anti-inflammatory, and anticancer properties. Nanoparticles are small particles that have a wide range of applications, including drug delivery, imaging, and diagnostics. The combination of phytochemicals and nanoparticles has the potential to provide several advantages over traditional drug delivery methods. Nanoparticles can improve the stability, solubility, and bioavailability of phytochemicals, making them more effective and easier to administer. Additionally, nanoparticles can target specific cells or tissues, which can improve the therapeutic index of phytochemicals.
The mechanism of action of phytochemical-loaded nanoparticles is not fully understood, but it is thought to involve several factors. First, nanoparticles can protect phytochemicals from degradation in the gastrointestinal tract, which can improve their bioavailability. Second, nanoparticles can target specific cells or tissues, which can increase the therapeutic index of phytochemicals. Third, nanoparticles can modulate the immune system, which can enhance the anti-cancer effects of phytochemicals (Pérez-De-Luque, 2017).
One of the key mechanisms by which phytochemical-loaded nanoparticles can improve the delivery of phytochemicals to cells is the enhanced permeability and retention (EPR) effect (Wu, 2021). The EPR effect is a phenomenon that occurs in tumors, where the tumor vasculature is leaky and the lymphatic drainage is impaired. This allows nanoparticles to accumulate in tumors while avoiding healthy tissues. The EPR effect is thought to be due to several factors, including the increased vascular permeability in tumors, the impaired lymphatic drainage in tumors, and the small size of nanoparticles. The EPR effect is effective in delivering a variety of drugs to tumors, including chemotherapeutic agents, imaging agents, and gene therapy vectors (Rao et al., 2016). Another mechanism by which phytochemical-loaded nanoparticles can improve the delivery of phytochemicals is by targeting specific cells or tissues (Pérez-De-Luque, 2017). This can be achieved by using nanoparticles that are coated with ligands that bind to specific receptors on the surface of cells like nanoparticles that are coated with antibodies can be targeted to cancer cells that express the corresponding antigen (Kim et al., 2021). Therapeutically, targeting phytochemical-loaded nanoparticles to specific cells or tissues can be used to improve the efficacy of treatment and reduce the side effects of treatment like nanoparticles that are targeted to cancer cells can be used to deliver chemotherapeutic agents directly to cancer cells, while avoiding healthy cells (Kim et al., 2021). This can help to reduce the side effects of chemotherapy, such as hair loss, nausea, and vomiting. Phytochemical-loaded nanoparticles can also modulate the immune system, which can enhance the therapeutic effects of phytochemicals including nanoparticles that are loaded with immune-stimulating agents can activate the immune system and promote the destruction of cancer cells (in case of cancer) and pathogenic cells (in case of infectious diseases). The immune system plays an important role in cancer therapy. The immune system can recognize and attack cancer cells, but it is often not effective in doing so. Nanoparticles that can modulate the immune system can help to improve the efficacy of cancer therapy by helping the immune system recognize and attack cancer cells more effectively. There has been a growing interest in the development of phytochemical-loaded nanoparticles for the treatment of cancer and other diseases (Rao et al., 2016).
Studies have revealed that the phytochemicals curcumin, hesperetin, quercetin, and broussochalcone A demonstrated half-maximum inhibitory concentration (IC50) values of 40μM, 60μM, 52.7μM and 88.1μM, respectively, against 3C-like protease. In the case of papain-like protease, the IC50 values for the phytochemicals curcumin, broussochalcone A, kaempferol, and quercetin were 5.7±0.3μM, 9.2μM, 16.3μM and 16.3μM, respectively (Dourado et al., 2021).
Phytochemical-loaded nanoparticles in COVID-19
Inorganic, lipid, and polymeric nanoparticles are being developed to combat SARS-CoV-2. The use of virus-shaped nanoparticles and lipid bilayers from the host cell are two successful antiviral techniques. There are techniques for the detection of SARS-CoV-2 nanoparticles, development of therapy for COVID-19 nanoparticles, and design of nanoparticle vaccinations for SARS-CoV-2. Nanotechnology plays a crucial role in combating COVID-19 (Rauf et al., 2022). It enables the development of rapid diagnostic tests using nanosensors for accurate and quick detection of the virus. Nanoparticles are utilized to design efficient antiviral coatings on surfaces, inhibiting the virus’s spread. Nanotechnology-based drug delivery systems deliver targeted treatments, including vaccines and antiviral drugs, with enhanced effectiveness. Nanoparticle-based vaccines stimulate a robust immune response, while nanocarriers transport antiviral drugs to the infected cells. Additionally, nanotechnology aids in the development of portable and wearable devices for continuous monitoring of vital signs and early detection of COVID-19 symptoms. By harnessing nanotechnology, powerful tools are developed to combat and control the spread of infectious diseases like COVID-19 (Prabhakar et al., 2023).
Nanoparticle-based detection is widely used which possesses the property to detect viral protein, viral RNA, and the detection of viral-specific antibodies. Gold nanoparticles coupled with SARS CoV- 2 polyclonal antibodies (Ventura et al., 2020) are used for immediate identification of the viral particle; additionally, these are coated with S protein glycans, which provide a flexible interpretation based on colorimetry techniques (Baker et al., 2020); tetramethylbenzidine PLGA nanoparticles are encoded by S protein-specific antibodies (Khoris, Ganganboina, Suzuki, & Park, 2021). These methods employ higher sensitivity and enhanced signal generation and parallel identification of numerous viral antigens, they can be easily handled generally strict conditions are not required. Gold nanoparticles with antisense oligonucleotides and fluorescent europium chelate nanoparticles with S9.6 antibodies aid in the diagnosis of the viral RNA segments (Moitra, Alafeef, Dighe, Frieman, & Pan, 2020; Wang et al., 2020). These can be visualized without any high-level apparatus, showing high accuracy, low adulteration risks, and rapid methods. The gold nanoparticles with coated with anti-human IgM antibodies and S proteins are used in the documentation of the virus-specific antibody, selenium nanoparticles with the viral nucleoproteins, lanthanide-doped polystyrene nanoparticles with anti-human IgG antibodies, and the Raman dye loaded silver nanoparticles with S proteins also aid in the same process (Chen et al., 2020; Huang, Wen, Shi, Zeng, & Jiao, 2020; Li et al., 2020; Wang et al., 2020). According to the principles of the lateral immune flow test, a small amount of blood can produce adequate results with a high sensitivity pattern.
Nanoparticle-based treatments have taken the lead over other medications lately due to advancements in the field of nanotherapeutics. They can be used as antiviral drug carriers, inducing direct death of viral particles and host mimicking for neutralization of viral processes. The antiviral drug carrier section mainly focuses on polymeric nanoparticles (Surnar, Kamran, Shah, & Dhar, 2020) (high drug load dimensions, measured release techniques), silica nanoparticles (Müller et al., 2016) (a comprehensive assortment of the ligands is possible), and lipid nanoparticles which are easily available and have proven therapeutic properties. For the effective killing of viral particles silver nanoparticles, ROS-generating nanoparticles and photothermal nanoparticles play an important role as these are significantly antiviral and exhibit broader activity against multiple viruses (Cai et al., 2022; Jeremiah, Miyakawa, Morita, Yamaoka, & Ryo, 2020; Khaiboullina, Uppal, Dhabarde, Subramanian, & Verma, 2020). Host-mimicking systems are recently developed and include the Lung epithelial cell nano sponge Macrophage Nano sponge and genetically engineered HEK-ACE2 cell Nano sponge. These can exhibit broader activities against multiple viruses and neutralize cytokine productions (Rao et al., 2020; Wang, Huang, Zhu, Tan, & Zhu, 2021).
Several vaccinations based on nanotechnological concepts are also being developed, including LNP-based mRNA vaccines encoding viral RBD or S protein (Saied, 2022; ) (Yang et al., 2020; Zhang et al., 2020), LNP-based mRNA vaccines with optimised coding sequences, and LNP-based mRNA vaccines with optimised coding sequences (Mckay et al., 2020; Zeng et al., 2020). VLP based on ferritin (Powell et al., 2021), and VLP based on computationally generated protein (Walls et al., 2020). Synthetic substrate-based VLP (Peng et al., 2020) are being used due to their quick synthesis, low immunisation dose, good half-life, immunomodulatory capabilities, non-communicable nature, arrangement of polyvalent antigens, as well as antigen and adjuvant administration in tandem.
The COVID-19 pandemic, which is brought on by the SARS-CoV-2 virus, has developed into a serious global health emergency that requires prompt access to effective treatments. Plants produce phytochemicals that have demonstrated promise in the fight against viruses, including coronaviruses. However, their poor stability and bioavailability make it challenging to use them in clinical settings, so that there has been a lot of interest in creating phytochemical-loaded nanoparticles as a solution to these issues. Studies have reported effective activity of numerous polyphenolic compounds like quercetin, myricetin, kaempferol, resveratrol and apigenin, against different strains of coronaviruses (Majnooni et al., 2020).
Alkaloids, naturally occurring organic compounds characterized by the presence of at least one nitrogen atom typically within a heterocyclic ring, constitute a diverse group of plant secondary metabolites. Several alkaloids have shown potential in inhibiting SARS-CoV-2 components or infection in vitro. Berberine, an alkaloid found in plants, has demonstrated inhibitory effects on SARS-CoV-2 entry, genome replication, and infectious virus production (Huang et al., 2021). A derivative of berberine called BE-33 exhibited even more potent activity against SARS-CoV-2, reducing viral loads and inflammatory cytokines in animal models (Xia et al., 2020). Berberine, another alkaloid, has been observed to reduce infectious virus production in cells. A screening of FDA-approved drugs identified alkaloids such as bromhexine, reserpine, hydroquinone, and quinidine as potential anti-SARS-CoV-2 agents (Ku et al., 2020). Quinacrine and quinine, which are antimalarial alkaloids, have also shown inhibitory effects against SARS-CoV-2 in vitro (Große et al., 2021). The iminosugar castanospermine and its prodrug celgosivir have demonstrated protective effects against SARS-CoV-2, reducing viral genome replication and S protein levels. Cepharanthine, hernandezine, neferine, and their analogs have shown inhibitory effects on SARS-CoV-2 entry and replication (Huang et al., 2021). Emetine has been reported to reduce SARS-CoV-2 replication in vitro and improve symptoms in COVID-19 patients. Homoharringtonine, lycorine, oxysophoridine, and tetrandrine have also shown inhibitory effects on SARS-CoV-2 replication or entry (Choy et al., 2020). Derivatives of alkaloids, such as isatin derivatives, topotecan, and tylophorine derivatives, have exhibited anti-SARS-CoV-2 effects, indicating the potential for optimizing alkaloid structures in the development of antiviral agents (Yang et al., 2020). Various studies have reported antiviral activity of alkaloids against SARS-CoV-2. This includes lycorine extracted from Lycoris radiata, 10-hydroxyusambarensine from Strychnos usambarensis, 6-oxoisoiguesterin and bisnorterperpenes from Salacia madagascariensis; and tetrandrine (at 0.33μM concentration), cepharanthine (at 0.83μM,concentration) bisbenzylisoquneyl and fangchinoline (at 1.01μM concentration) from Stephania tetrandra (Majnooni et al., 2020).
Flavonoids, a large and structurally diverse group of secondary metabolites produced by plants, have shown activity against SARS-CoV-2 infection in vitro. Baicalin and baicalein have been observed to inhibit SARS-CoV-2 infection and reduce lung damage in animal models. Baicalin may also inhibit the endoribonuclease activity of SARS-CoV-2 NSP15 (Yang et al., 2020). Analogs of baicalein, such as dihydromyricetin, myricetin, scutellarein, and quercetagetin, have demonstrated inhibitory effects on viral replication (Liu et al., 2021). Brazilin has been shown to inhibit SARS-CoV-2 pseudovirus entry, possibly by inhibiting the interaction between the viral spike protein and ACE2 receptors (Goc, Sumera, Rath, & Niedzwiecki, 2021). Isorhamnetin and panduratin A have also exhibited inhibitory effects on SARS-CoV-2 infection in vitro. Chrysanthemum, herbacetin, kaempferol, pectolinarin, and quercetin have demonstrated the capacity to inhibit SARS-CoV-2 Mpro, an essential viral enzyme (Pitsillou et al., 2020). Rutin, a rutinose-bound form of quercetin, has been found to inhibit the deubiquitinase activity of another viral enzyme, Plpro (Pitsillou et al., 2020). Naringenin has shown protective effects against SARS-CoV-2-induced cytopathic effects and has been suggested to inhibit Mpro and TPC2 (Abdallah et al., 2021). Flavonoids from Camellia sinensis, such as epicatechin-3-gallate (EGCG) and theaflavin-3,3’-di-O-gallate (TF3), have displayed virucidal effects on SARS-CoV-2 particles (Xu, Xu, & Zheng, 2017). They have also shown inhibitory effects on viral binding, entry, and Mpro activity. EGCG has additionally been observed to inhibit the endoribonuclease activity of NSP15 (Hong et al., 2021), while TF3 may downregulate cathepsin L levels, contributing to its inhibitory effects on viral entry. Flavonoids exhibit a broad range of pharmacological activities against SARS-CoV-2, including inhibitory effects on viral entry, replication, and key viral enzymes (Yapasert, Khaw-On, & Banjerdpongchai, 2021). Studies have identified inhibition of activity of papain-like proteases, which are important for the propagation of SARS-CoV-2 by extracts of Paulownia tomentosa attributed to the presence of flavonoids like tomentin A-E. The flavonoids, apigenin-7-O-rhamnoglucoside, pectolinarin and herbacetin at 20μM concentration from Paulownia tomentosa and 10 polyphenols including papyriflavonol A at 3.7μM concentration from Broussonetia papyrifera were reported to inhibit the activity of the enzyme 3C-like protease, responsible for replication of SARS-CoV-2. The flavonoid, theaflavin obtained from Camella sinensis inhibiṭed the activity of RNA-dependent RNA Polymerase of SARS-CoV-2 Studies reveal that the phytocompound, hesperidin, isolated from citrus is a potential clinical trial candidate for development of therapeutics against SARS-CoV-2 (Majnooni et al., 2020).
Terpenes and terpenoids, which are part of the largest family of plant secondary metabolites, have shown anti-SARS-CoV-2 activity in vitro. Andrographolide, a diterpenoid, has been reported to inhibit viral production and late stages of infection. Diterpenes such as cryptotanshinone, tanshinone I, tanshinone II, and dihydrotanshinone I have demonstrated the ability to inhibit Plpro, an essential viral enzyme (Zhao et al., 2021). Triterpenoids, including (Alhadrami, Sayed, Sharif, Azhar, & Rateb, 2021). Glycosides like glycyrrhizin and platycodin D, classified as triterpene glycosides or saponins, have exhibited anti-SARS-CoV-2 activity by inhibiting viral production and Mpro (Mieres-Castro & Mora-Poblete, 2023)viral entry. Clinical trials with curcumin formulations have shown potential benefits in COVID-19 patients, including decreased pro-inflammatory cytokines, lower mortality rates, and accelerated symptom recovery (Amalraj, Pius, Gopi, & Gopi, 2017). Other phytochemicals, such as cardiac glycosides (digoxin and ouabain), glycosides (sennoside B and acetonide), polyphenols (gingerol, resveratrol, pterostilbene), and lignans (dyphylline, nordihydroguaiaretic acid), have demonstrated inhibitory effects on viral replication, entry, and key viral enzymes (Wolff & Veit, 2021). The structural complexity of phytochemicals has posed challenges in their production and synthesis on an industrial scale. However, advancements in synthetic chemistry and biotechnology have allowed for the synthesis or semi-synthesis of complex natural products. Drug delivery technologies, such as nano-formulations, have improved the bioavailability and pharmacological profiles of phytochemicals. Continued research and optimization of plant-derived compounds, along with advancements in compound optimization and biosynthesis, may address limitations and expand the clinical application of phytochemicals. Plants offer a vast array of potential candidates for the treatment of COVID-19 and other viral diseases. Efforts should be made to identify phytochemicals with broad-spectrum antiviral potential to optimize semi-synthetic and biosynthetic techniques (Españo, Kim, Lee, & Kim, 2021). Concerted efforts are needed to maximize resources and develop treatment agents to mitigate the impact of viral outbreaks in the future.
A considerable amount of preclinical research has been conducted on utilizing phytocompounds for their therapeutic benefits. However, majority of the cases have exhibited clinical failure in translation of phytochemicals for therapeutic use by humans due to inefficiency in delivering the phytocompounds to the target site. This limitation can be overcome by using nanoparticle-based carriers to facilitate targeted delivery. The nanoformulations of phytocompounds can be developed using common nanoparticle carriers like micelles, polymeric dendrimers, nanoemulsions, metallic nanoparticles and liposomes. The nanoparticle carriers also protect by pharmaceutic molecule from the external environment providing extended systemic circulation (Chavda et al., 2023). The combination of nanoparticles with phytocompounds results in improved antimicrobial activity due to enhanced mechanical, biopharmaceutical, physicochemical, morphological, release and bioavailability characteristics. The phytochemicals can be used in combination with drugs like antibiotics resulting in boosted efficacy with reduced concentration of antibiotics required for the treatment. They also enhance the immunity providing protection from wide array of diseases consequently improving the health of people. This approach can also be utilized against antibiotic resistant bacteria (Díaz-Puertas, Álvarez-Martínez, Falco, Barrajón-Catalán, & Mallavia, 2023). The domain of nanotechnology has transformed management of infectious and non-infectious diseases by facilitating targeted drug release improving the health of patients (Chavda et al., 2023).
Figure 2 provides an overview of the phytochemical nanoparticles used for the management of COVID-19.
Figure 2
Overview of phytochemical loaded nanoparticles for COVID-19 management(created using biorender.com)
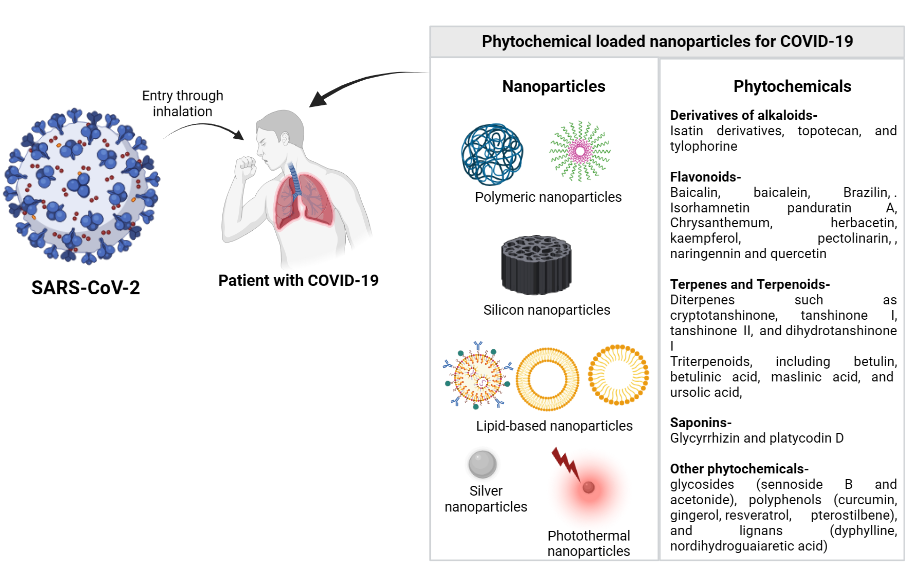
The first step in creating phytochemical-loaded nanoparticles is to select phytochemicals that have been demonstrated to be effective against SARS-CoV-2. Numerous studies have discovered phytochemicals that could obstruct viral entry, replication, or the function of essential viral enzymes. Some examples include terpenes and terpenoids like andrographolide and glycyrrhizin, alkaloids like berberine and berberine, flavonoids like baicalin and curcumin, and polyphenols like resveratrol and EGCG. These phytochemicals are the active components that will be inserted inside the nanoparticles. The following step is to select the best nanoparticle formulations to incorporate the phytochemicals. Common types of nanoparticle systems include liposomes, polymeric nanoparticles, solid lipid nanoparticles, and lipid-based nanocarriers. The stability, capacity, method of release, and destination of a nanoparticle system are all important considerations. Phytochemicals can be enclosed in a variety of ways before being added to nanoparticles. Some of these techniques include solvent evaporation, emulsion/solvent diffusion, nanoprecipitation, and supercritical fluid methods. Regarding effectiveness, scalability, and the physical and chemical characteristics of the nanoparticles it produces, each method has advantages and disadvantages. Characterizing the phytochemical-loaded nanoparticles is crucial to ensuring their high quality, stability, and efficacy. Particle size, shape, surface charge, encapsulation effectiveness, drug release rate, and stability are important to consider. Such techniques as dynamic light scattering, electron microscopy, zeta potential analysis, encapsulation efficiency assessment, and drug release studies are frequently used for characterization. Both in vitro and in vivo studies are used to assess the efficacy of the phytochemical-loaded nanoparticles in combating viruses. In vitro, studies employ cell-based assays to determine how they impact viral replication, entry, or the blocking of viral enzymes. Animal models such as SARS-CoV-2-infected mice or hamsters are used to test the effectiveness of the nanoparticles as treatments, how they operate within the body, and whether they are safe. There are many waysto enhance the precise targeting and absorption of nanoparticles. These include altering nanoparticle surfaces with ligands or antibodies that are particular to viral receptors or infected cells. Additionally, phytochemicals may only be released by stimuli-responsive nanoparticle systems at the site of an infection or in response to specific triggers like pH or temperature changes (Mohanty, Sahoo, Paidesetty, & Padhy, 2023).
Table 1 represents the details of some phytochemical-loaded nanoparticles developed for COVID-19.
Table 1
Phytochemical loaded nanoparticles for COVID-19
Sl.No. | Phytochemical | Nanoparticle | Country | Mechanism of action | Advantages | Disadvantages | Reference |
1. | Curcumin | Gold nanoparticles | India | Inhibits the growth of SARS-CoV-2-infected cells by targeting multiple pathways, including cell cycle arrest, apoptosis, and autophagy. Curcumin, a compound found in turmeric, has antiviral activity against SARS-CoV-2. It works by binding to the spike protein of the virus, preventing it from attaching to and entering cells. It also interferes with the activity of the virus’s RNA polymerase, an enzyme that is essential for the virus to replicate. Additionally, curcumin can induce apoptosis, or programmed cell death, in cells that are infected with SARS-CoV-2. This helps to prevent the virus from spreading to other cells. | Enhanced solubility and stability of curcumin. | Potential for site-specific delivery of curcumin. Low bioavailability, short half-life, Potential interactions with medications | |
2. | Resveratrol | Liposomes | USA | Inhibits the replication of SARS-CoV-2 by targeting the virus’s RNA polymerase. Resveratrol, a compound found in grapes, red wine, and peanuts, has antiviral activity against SARS-CoV-2. It works by binding to the spike protein of the virus, preventing it from attaching to and entering cells. It also interferes with the activity of the virus’s RNA polymerase, an enzyme that is essential for the virus to replicate. Additionally, resveratrol can induce apoptosis, or programmed cell death, in cells that are infected with SARS-CoV-2. This helps to prevent the virus from spreading to other cells. | Enhanced solubility and stability of resveratrol. | Potential for site-specific delivery of resveratrol Low bioavailability . | Ellen et al. (2021); Pasquereau et al. (2021); Yang et al. (2021) |
3. | Apigenin | Polymeric nanoparticles | China | Inhibits the entry of SARS-CoV-2 into cells by targeting the virus’s spike protein. Apigenin, a compound found in chamomile, parsley, and citrus fruits, has antiviral activity against SARS-CoV-2. It works by binding to the spike protein of the virus, preventing it from attaching to and entering cells. It also interferes with the activity of the virus’s RNA polymerase, an enzyme that is essential for the virus to replicate. Additionally, apigenin can induce apoptosis, or programmed cell death, in cells that are infected with SARS-CoV-2. This helps to prevent the virus from spreading to other cells. | Enhanced solubility and stability of apigenin. | Potential for site-specific delivery of apigenin. | |
4. | EGCG | Carbon nanotubes | China | Inhibits the replication of SARS-CoV-2 by targeting the virus’s RNA polymerase. EGCG, or epigallocatechin-3-gallate, is a compound found in green tea. It has antiviral activity against SARS-CoV-2. It works by binding to the spike protein of the virus, preventing it from attaching to and entering cells. It also interferes with the activity of the virus’s RNA polymerase, an enzyme that is essential for the virus to replicate. Additionally, EGCG can induce apoptosis, or programmed cell death, in cells that are infected with SARS-CoV-2. This helps to prevent the virus from spreading to other cells. | Enhanced solubility and stability of EGCG. | Potential for site-specific delivery of EGCG. | |
5. | Quercetin | Gold nanoparticles | India | Inhibits the growth of SARS-CoV-2-infected cells by targeting multiple pathways, including cell cycle arrest, apoptosis, and autophagy. Quercetin is a naturally occurring flavonoid found in many fruits and vegetables. It has antiviral activity against SARS-CoV-2. It works by binding to the spike protein of the virus, preventing it from attaching to and entering cells. It also interferes with the activity of the virus’s RNA polymerase, an enzyme that is essential for the virus to replicate. Additionally, quercetin can induce apoptosis, or programmed cell death, in cells that are infected with SARS-CoV-2. This helps to prevent the virus from spreading to other cells. | Enhanced solubility and stability of quercetin. | Potential for site-specific delivery of quercetin. | |
6. | Gingerol | Liposomes | China | Inhibits the replication of SARS-CoV-2 by targeting the virus’s RNA polymerase. Gingerol is a compound found in ginger. It has antiviral activity against SARS-CoV-2. It works by binding to the spike protein of the virus, preventing it from attaching to and entering cells. It also interferes with the activity of the virus’s RNA polymerase, an enzyme that is essential for the virus to replicate. Additionally, gingerol can induce apoptosis, or programmed cell death, in cells that are infected with SARS-CoV-2. This helps to prevent the virus from spreading to other cells. | Enhanced solubility and stability of gingerol. | Potential for site-specific delivery of gingerol. | |
7. | EGCG | Polymeric nanoparticles | USA | Inhibits the entry of SARS-CoV-2 into cells by targeting the virus’s spike protein. EGCG, or epigallocatechin-3-gallate, is a compound found in green tea. It has antiviral activity against SARS-CoV-2. It works by binding to the spike protein of the virus, preventing it from attaching to and entering cells. It also interferes with the activity of the virus’s RNA polymerase, an enzyme that is essential for the virus to replicate. Additionally, EGCG can induce apoptosis, or programmed cell death, in cells that are infected with SARS-CoV-2. This helps to prevent the virus from spreading to other cells. | Enhanced solubility and stability of EGCG. | Potential for site-specific delivery of EGCG. | |
8. | Ursolic acid | Carbon nanotubes | India | Inhibits the replication of SARS-CoV-2 by targeting the virus’s RNA polymerase. Ursolic acid is a compound found in fruits, vegetables, and herbs. It has antiviral activity against SARS-CoV-2. It works by binding to the spike protein of the virus, preventing it from attaching to and entering cells. It also interferes with the activity of the virus’s RNA polymerase, an enzyme that is essential for the virus to replicate. Additionally, ursolic acid can induce apoptosis, or programmed cell death, in cells that are infected with SARS-CoV-2. This helps to prevent the virus from spreading to other cells. | Enhanced solubility and stability of Ursolic acid. | Potential for site-specific delivery of Ursolic acid. | (Al-Kuraishy, Al-Gareeb, El-Saber, & Batiha, 2022; Al-Kuraishy, Al-Gareeb, Negm, Alexiou, & Batiha, 2022) |
9. | Berberine | Quantum dots | China | Inhibits the growth of SARS-CoV-2-infected cells by targeting multiple pathways, including cell cycle arrest, apoptosis, and autophagy. Berberine is a compound found in plants such as goldenseal and barberry. It has antiviral activity against SARS-CoV-2. It works by binding to the spike protein of the virus, preventing it from attaching to and entering cells. It also interferes with the activity of the virus’s RNA polymerase, an enzyme that is essential for the virus to replicate. Additionally, berberine can induce apoptosis, or programmed cell death, in cells that are infected with SARS-CoV-2. This helps to prevent the virus from spreading to other cells. | Enhanced solubility and stability of berberine. | Potential for site-specific delivery of berberine. | |
10. | Naringenin | Gold nanoparticles | China | Inhibits the replication of SARS-CoV-2 by targeting the virus’s RNA polymerase Naringenin is a flavonoid found in many fruits and vegetables, including citrus fruits, grapes, and onions. It has antiviral activity against SARS-CoV-2. It works by binding to the spike protein of the virus, preventing it from attaching to and entering cells. It also interferes with the activity of the virus’s RNA polymerase, an enzyme that is essential for the virus to replicate. Additionally, naringenin can induce apoptosis, or programmed cell death, in cells that are infected with SARS-CoV-2. This helps to prevent the virus from spreading to other cells. | Enhanced solubility and stability of naringenin | Enhanced solubility and stability of naringenin. | Clementi et al. (2021); Tutunchi, Naeini, Ostadrahimi, and Hosseinzadeh-Attar (2020) |
11. | Pterostilbene | Carbon nanotubes | China | Inhibits the replication of SARS-CoV-2 by targeting the virus’s RNA polymerase Pterostilbene is a polyphenol found in grapes, blueberries, and peanuts. It has antiviral activity against SARS-CoV-2. It works by binding to the spike protein of the virus, preventing it from attaching to and entering cells. It also interferes with the activity of the virus’s RNA polymerase, an enzyme that is essential for the virus to replicate. Additionally, Pterostilbene can induce apoptosis, or programmed cell death, in cells that are infected with SARS-CoV-2. This helps to prevent the virus from spreading to other cells. | Enhanced solubility and stability of Pterostilbene | Enhanced solubility and stability of Pterostilbene |
The nanoparticle-based therapeutics and vaccines are widely being studied in pre-clinical and clinical settings. Many metallic nanoparticle-based therapeutics have been reported to exhibit antiviral activity by inhibiting the viral replication. Silicon nanoparticles are found to be efficient in scavenging viruses and has the potential to be used in the treatment of COVID-19. Iron oxide nanoparticles can potentially be used in therapeutic regime for COVID-19. It can bind with the S1-RBD receptor that is used for attachment to host by SARS-CoV-2. Additionally, zinc oxide, silver, copper, and grapheme oxide can be used for targeted inhibition of viral transmission and propagation. FDA has approved the use of polymer-based, non-polymer based and lipid based as well as nanocrystals in drug delivery, while the metallic, protein-based and inorganic nanoparticles are awaiting approval. Presently, there are around 26 nanoparticle-based vaccines in clinical trials and over 60 are in pre-clinical phase (Rauf et al., 2022). The nanoparticles prevalent in FDA approved nanoparticle-based therapeutics are polymeric, liposomal and lipid-based in the percentages 29%, 22% and 21%, respectively (Namiot, Sokolov, Chubarev, Tarasov, & Schiöth, 2023). With the increasing research on pharmacological phytochemicals for COVID-19, currently there are various phytochemicals in clinical trials. Many of the trials were initiated in 2020. The list includes polyphenols (NCT04400890), resveratrol (NCT0452993, NCT04536090 and NCT04377789), artemisinin-curcumin (NCT04382040), hesperidin-diosmin (NCT04452799), quercetin (NCT04468139 and NCT04377789), colchicine (NCT04527562, NCT04392141, NCT04375202, NCT04360980 and NCT04355143), epigallocatechin gallate (NCT04446065), tetrandrine (NCT04308317), glycyrrhizin (NCT04487964) and berberine (NCT04479202) (Majnooni et al., 2020).
A clinical trial analyzing the supplementation of sunflower lecithin with adjuvant quercetin, demonstrated improvement in symptoms of patients in the early stage of COVID-19 infection. It also inhibited the development of severe infection when used in combination with other drugs in treatment regime. The results of the study confirmed quicker clearance of SARS-CoV-2 preventing further progression of the disease. The phytochemical baicalein and its natural analogues like myricetin, scutellarein, quercetagetin and dihydromyricetin exhibited inhibition of SARS-CoV-2 in the in-vitro studies. The clinical trial on the formulation of luteolin was demonstrated to be advantageous for patients with brain fog and chemo fog due to long-COVID syndrome. A randomized clinical trial exhibited reduction in the symptoms of COVID-19 on using the phytochemical hesperidin. In another clinical study on antiviral activity of curcumin, it was found that the administration of nano-curcumin capsule orally could significantly reduce mRNA expression of pro-inflammatory cytokines (Das, Khan, Roy, & Das, 2023). The phytochemical silymarin, effective against SARS-CoV-2, is in Phase 3 of clinical trial (NCT04394208) and is in the recruitment stage. It focuses on patients, particularly adults, with COVID-19 pneumonia. Silymarin when used as silymarin/curcumin loaded BSA nanoparticles, exhibited anti-inflammatory and anti-oxidant properties, consequently protecting lungs of patients infected with SARS-CoV-2 (Lei, Chen, Wu, Duan, & Men, 2022).
Advantages of Phytochemical-loaded nanoparticles in COVID-19 management
In contrast to their previous status as relatively harmless respiratory pathogens, the emergence of SARS and MERS highlighted the significance of coronaviruses as respiratory tract pathogens capable of causing severe diseases like acute respiratory distress syndrome (ARDS) (Majnooni et al., 2020). Human coronaviruses (HcoVs) have single-stranded RNA genomes and encode various nonstructural proteins, structural proteins, and accessory proteins, which differ among different strains. Clinical symptoms of COVID-19, caused by SARS-CoV-2, include fever, cough, dyspnea, fatigue, headache, myalgia, and diarrhea. Some patients progress to experience shortness of breath and persistent or recurrent fever, with a subset requiring intensive care treatment (Merad & Martin, 2020). The exact pathobiology and molecular mechanisms underlying coronavirus-associated lung injury, including COVID-19, are not fully understood. However, several key molecular mediators such as TNF-α, IL-1, IL-6, IL-8, IL-1β, NF-κB, MMPs, MAPK, COX-2, ROS, iNOS, Nrf2, autophagy-related molecules, JAK/STATs pathway, ERK, Akt, and ACE2 receptor have been implicated in the pathogenesis of COVID-19 and associated lung injury. Dysregulation of the ACE2 receptor, which serves as an entry point for SARS-CoV-2 into lung cells, has received significant attention. Dysregulation of ACE2 and the renin-angiotensin system can lead to increased inflammation, vascular permeability, and cardiovascular/lung complications (Bellik, Hammoudi, Abdellah, Iguer-Ouada, & Boukraâ, 2012; Favarin, Oliveira, De, Oliveira, & Rogerio, 2013). Pathological features observed in SARS patients include edematous lungs, multinucleated cells, atypical enlarged pneumocytes, squamous metaplasia of bronchial and alveolar epithelial cells, fibrogranulative tissue multiplication, vascular injury, apoptosis, gastrointestinal and CNS infections, and involvement of other organs such as the spleen, lymph nodes, liver, thyroid glands, skeletal muscle, and adrenal medulla. Phytochemicals loaded with nanoparticles can offer several advantages specifically in the context of COVID-19:
• Enhanced Antiviral Activity: Phytochemicals loaded with nanoparticles can exhibit enhanced antiviral activity against SARS-CoV-2, the virus responsible for COVID-19. The combination of phytochemicals and nanoparticles can potentially increase the efficacy of these compounds in inhibiting viral replication and reducing viral load (Ben-Shabat, Yarmolinsky, Porat, & Dahan, 2020).
• Targeted Delivery to the Respiratory System: By encapsulating phytochemicals within nanoparticles, targeted delivery to the respiratory system can be achieved. This is particularly advantageous for COVID-19, as the primary site of infection is the respiratory tract. Targeted delivery can maximize the concentration of phytochemicals at the site of viral replication, potentially leading to improved therapeutic outcomes (Subramanian et al., 2016).
• Controlled Release in the Lungs: Nanoparticles can be engineered to release phytochemicals in a controlled manner within the lungs. This sustained release can ensure a prolonged presence of the active compounds, allowing for continuous antiviral effects and potentially reducing the need for frequent dosing (Devkota, A, & D, 2022).
• Protection of Phytochemicals: Nanoparticles protect phytochemicals from degradation and enzymatic activity. This protection can enhance the stability and integrity of the loaded compounds, ensuring their potency and effectiveness against SARS-CoV-2 (Yousefi, Narmani, & Jafari, 2020).
• Combination Therapy Potential: Nanoparticles can facilitate the delivery of multiple phytochemicals or even other therapeutic agents, enabling combination therapy for COVID-19. This approach can target different stages of the viral lifecycle, enhance antiviral synergy, and potentially overcome drug resistance (Aw-Yong et al., 2018).
• Reduced Systemic Toxicity: By encapsulating phytochemicals within nanoparticles, the potential for systemic toxicity can be minimized. The controlled release and targeted delivery of loaded phytochemicals can help reduce off-target effects and enhance safety profiles (Aman, Hashim, & Meshali, 2018)
• Potential for Inhalation Delivery: Nanoparticles loaded with phytochemicals can be designed for inhalation delivery, allowing direct administration to the respiratory system. Inhalation delivery can improve the local concentration of phytochemicals in the lungs and facilitate their interaction with SARS-CoV-2 (Al-Hatamleh et al., 2020).
It’s important to note that research in this area is still ongoing, and the clinical application of phytochemicals loaded with nanoparticles for COVID-19 treatment is being actively investigated. Further studies and clinical trials are needed to fully understand the efficacy, safety, and optimal utilization of this approach in combating COVID-19.
CONSTRAINTS
The lack of standardization in formulation and preparation techniques is a significant barrier to the use of phytochemical-loaded nanoparticles for COVID-19 control. With respect to plant source, extraction technique, and nanoparticle synthesis method, particle size, surface charge, drug encapsulation effectiveness, and stability can vary. The development of this therapeutic strategy is hampered by the absence of standardized protocols because it makes it more difficult to compare and replicate studies. The pharmacokinetics and biodistribution of phytochemical-loaded nanoparticles must be understood to be used effectively. These nanoparticles need to be designed so that they can function in the respiratory system, where SARS-CoV-2 is most prevalent (Bezbaruah et al., 2022). However, the size, surface charge, and hydrophobicity of nanoparticles can have an impact on their distribution, metabolism, and excretion (Sun, Jiang, Wu, Bai, & Zhai, 2019; Xu et al., 2010). Achieving ideal pharmacokinetic profiles and maintaining sufficient concentrations at the infection site are both difficult tasks. Due to stability issues, phytochemical-loaded nanoparticles may only be effective and have a short shelf life. Storage, delivery, and even administration are all potential times for accumulation, drug leakage, and degradation. To enhance nanoparticle stability and maintain their integrity from synthesis to clinical application, suitable formulation strategies must be created. Even though phytochemicals themselves are generally regarded as safe, their potential toxicity is increased when they are encapsulated in nanoparticles (Shae, Postma, & Wilson, 2016). Nanoparticles have a wide range of unfavorable side effects, including immune reactions, organ toxicity, and oxidative stress, depending on their characteristics and dosage. Before these nanosystems are applied in the clinic, extensive toxicity evaluations are required to pinpoint dangers and enhance their safety. Effective management of COVID-19 requires efficient and precise targeting of phytochemical-loaded nanoparticles to the site of action, such as SARS-CoV-2 infected lung tissue. High levels of targeting efficiency and specificity are still hard to attain, though. Particle size, surface modifications, and the presence of biological barriers can all affect how well nanoparticles reach and enter target cells or tissues. We must carefully consider the design and conduct additional research on the most effective targeting techniques to overcome these obstacles. There are many difficulties when producing phytochemical-loaded nanoparticles at an industrial scale from lab-scale synthesis. Three essential elements for a successful translation are scalability, reproducibility, and cost-effectiveness. To meet the demands of widespread COVID-19 management, it is essential to develop scalable, dependable manufacturing processes. Novel therapeutics must navigate a difficult regulatory environment, especially if they use nanoparticles. To assess the quality, safety, and efficacy of phytochemical-loaded nanoparticles, appropriate regulatory frameworks are required. The expense and effort necessary to comply with these regulations may cause a delay in the release of the therapeutic option (Rai et al., 2021).
Conclusion and Future Prospects
There is a growing interest in the use of phytochemical-loaded nanoparticles for the treatment of COVID-19. These facilitate reliable, targeted delivery of the drug molecules (phytochemicals) to the site of infection, as well as increased solubility and stability of the phytochemicals. As the virus gets mutated frequently it is difficult for its treatment and the formulation of vaccines. Several phytochemicals have been shown to have antiviral properties, including curcumin, quercetin, resveratrol, and EGCG (Dinda et al., 2023; Ellen et al., 2021; Raghav et al., 2022; Zahedipour et al., 2020). These phytochemicals can inhibit the replication of viruses by interfering with their ability to enter cells, replicate their genetic material, or assemble new virions (Españo et al., 2021).
Phytochemicals showing antiviral properties, and nanoparticles can improve the delivery of phytochemicals to cells. This can potentially help to inhibit the replication of the SARS-CoV-2 virus and reduce the severity of the disease. Second, nanoparticles can be targeted to specific cells or tissues, such as the lungs or the immune system. This can help to deliver phytochemicals to the areas where they are needed most, and it can also help to reduce the side effects of treatment. Thirdly, nanoparticles can be used to deliver multiple phytochemicals at the same time. This can provide a more comprehensive treatment for COVID-19 and it can also help to reduce the risk of drug resistance. According to the research conducted by (Saleh et al., 2023) aimed at proving the antiviral activity of PVA/PLGA NPs loaded with CGA against low pathogenic human coronavirus (HcoV-229E) and MERS-CoV (NRCEHKU270) compared to free CGA.
The use of phytochemical-loaded nanoparticles for the treatment of COVID-19 is still in its early stages, and several challenges need to be addressed before they can be widely used. One challenge is to develop stable nanoparticles that can deliver phytochemicals to cells in a targeted manner. Nanoparticles can be easily degraded in the body, and they can also be taken up by the wrong cells (Badshah et al., 2021). This can reduce the effectiveness of treatment and can also lead to side effects. Another challenge is to conduct more research to assess the safety and efficacy of phytochemical-loaded nanoparticles in humans (Asif, Saleem, Saadullah, Yaseen, & Zarzour, 2020). There have been several animal studies, but more research is needed to determine the safety and efficacy of these nanoparticles in humans. Finally, there is a need to develop methods for manufacturing phytochemical-loaded nanoparticles at a large scale. This is a challenge because the manufacturing process can be complex and expensive. Despite these challenges, the potential benefits of phytochemical-loaded nanoparticles offer promising treatment options for COVID-19 on further research and development.